UV-Visible Spectroscopy: Principle
UV-Visible spectroscopy, also known as electronic spectroscopy, is a technique used to measure the absorption of ultraviolet (UV) and visible light by a substance. The principle behind this technique is based on the absorption of light by molecules, which causes the promotion of electrons from lower energy levels (ground state) to higher energy levels (excited state). This typically involves the excitation of electrons in molecular orbitals, such as from the Highest Occupied Molecular Orbital (HOMO) to the Lowest Unoccupied Molecular Orbital (LUMO).
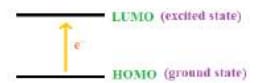
The amount of light absorbed depends on the structure of the molecule, particularly the extent of conjugation (delocalized π electrons) in the molecule. More conjugated systems have smaller energy gaps between the HOMO and LUMO, allowing them to absorb longer wavelengths of light.
The wavelength range for:
- UV light: 200-400 nm
- Visible light: 400-800 nm
Lambert-Beer's Law
Lambert-Beer's Law (also known as Beer's Law) is the fundamental principle governing UV-Visible spectroscopy. It describes how the absorption of light by a sample is related to the concentration of the absorbing species and the path length through which the light passes.
Lambert-Beer's Law Equation
Where:
- = absorbance (no units, since it's a logarithmic measure)
- = molar absorptivity or molar extinction coefficient (L mol⁻¹ cm⁻¹), a constant specific to the substance and wavelength of light
- = concentration of the sample (mol L⁻¹)
- = path length (cm), typically the length of the cuvette containing the sample
Alternatively, if you know the absorbance and wish to calculate the molar absorptivity, the equation can be rearranged as:
Some Important Terms:
-
Absorbance (A) is directly proportional to the concentration of the absorbing species and the path length .
-
Molar absorptivity is a constant that indicates how strongly a substance absorbs light at a particular wavelength. Higher values of mean greater absorbance.
-
Absorbance: The absorbance is related to the intensity of the incident light and transmitted light :
Where is the intensity of the incident light and is the intensity of the transmitted light.
-
Transmittance (T) Transmittance refers to the fraction of incident light that passes through the sample. It is defined as:
Where:
- = transmittance
- = intensity of the incident light
- = intensity of the transmitted light
Transmittance and absorbance are related by the following equation:
Instrumentation of UV Spectroscopy
Instrumentation refers to the equipment and devices used to perform UV spectroscopy. The typical setup consists of several key components:
-
Light Source:
- Deuterium Lamp: Typically used for the UV range (160-400 nm).
- Tungsten Lamp: Used for the visible range (400-800 nm).
- The light source generates the UV and visible light necessary for the measurement.
-
Monochromator - A device that isolates specific wavelengths of light from the light source. It usually consists of prisms or diffraction gratings that disperse light into its component wavelengths, allowing for the selection of a specific wavelength for measurement.
-
Sample Holder - Often a cuvette (usually made of quartz or glass) that holds the sample solution. The path length of the cuvette is a critical factor in the measurement of absorbance.
-
Detector - Photodiodes or Photomultiplier Tubes (PMTs) are commonly used detectors that convert the transmitted light into an electrical signal. This signal is then processed to determine the absorbance of the sample.
-
Data Processing System - Software and electronic systems are used to process the signals from the detector, displaying absorbance spectra and allowing for quantitative analysis.
Electronic Transitions
When a molecule absorbs electromagnetic radiation in the UV-visible region, its electrons are promoted from the ground state to an excited state or from bonding orbitals to anti-bonding orbitals.
Types of Electronic Transitions
-
σ → σ* Transition: This is the transition of an electron from a bonding sigma (σ) orbital to an anti-bonding sigma (σ*) orbital, represented as a σ → σ* transition. An example of this is in alkanes, where all atoms are connected by sigma bonds.
-
n → σ* & n → π* Transition: These transitions occur when an electron from a non-bonding molecular (n) orbital is promoted to either an anti-bonding sigma (σ*) orbital or an anti-bonding pi (π*) orbital, represented as n → σ* or n → π* transitions, respectively. These transitions require less energy than σ → σ* transitions. Examples include alkyl halides, aldehydes, and ketones.
-
π → π* Transition: This type of transition occurs in unsaturated molecules such as alkenes, alkynes, aromatic compounds, and carbonyl groups. The energy required for this transition is lower than that for an n → σ* transition.
Energy order of transitions:
Absorption, Intensity Shifts, and UV Spectrum
-
Bathochromic Shift (Red Shift): This refers to a shift in absorption towards longer wavelengths (higher λmax).
-
Hypsochromic Shift (Blue Shift): This refers to a shift in absorption towards shorter wavelengths (lower λmax).
-
Hyperchromic Shift: This occurs when the intensity of the absorption maximum (εmax) increases.
-
Hypochromic Shift: This occurs when the intensity of the absorption maximum (εmax) decreases.
Auxochromes
-
An auxochrome is a group of atoms attached to a chromophore that modifies the ability of the chromophore to absorb light, often by shifting the absorption wavelength or increasing the intensity of absorption.
-
Auxochromes do not absorb UV or visible light by themselves but influence the absorption properties of the chromophore when attached.
-
Auxochromes typically contain non-bonding electrons (lone pairs) and include functional groups like -OH (hydroxyl), -NH₂ (amino), -OR (alkoxy), and -SH (thiol).
-
The attachment of an auxochrome can cause:
- Bathochromic shift (Red shift): A shift to longer wavelengths (lower energy).
- Hyperchromic effect: An increase in the intensity of absorption.
Example: When a hydroxyl group (-OH) is attached to benzene (which is a chromophore), it shifts the absorption maximum to a longer wavelength.
Chromophores
-
A chromophore is a part of a molecule responsible for its color. It absorbs light in the UV or visible region and promotes an electron from the ground state to an excited state.
-
Chromophores contain pi (π) electrons or non-bonding (n) electrons that can absorb energy in the form of UV or visible light, leading to electronic transitions like π → π* or n → π*.
-
Common chromophores include C=C (double bonds), C=O (carbonyl groups), N=N (azo groups), NO₂ (nitro groups), etc.
Example: In molecules like benzene, the conjugated double bonds act as a chromophore and absorb UV light.
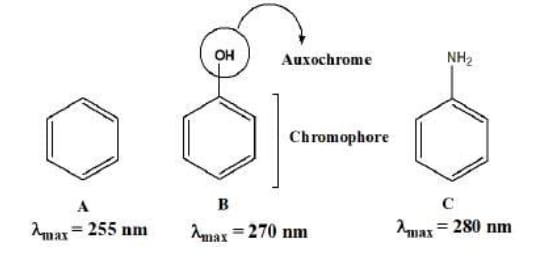
-
Structure A (Benzene): This is a simple aromatic molecule with a chromophore, and its maximum absorption wavelength (λmax) is 255 nm.
-
Structure B (Phenol - OH group attached): The hydroxyl group (-OH) is an auxochrome. When attached to the chromophore (benzene), it causes a bathochromic shift (red shift), increasing the λmax to 270 nm. This happens because the -OH group has lone pairs that interact with the π-electrons of the benzene ring, stabilizing the excited state and lowering the energy gap.
-
Structure C (Aniline - NH₂ group attached): The amino group (-NH₂) is another auxochrome. It causes an even larger bathochromic shift compared to the hydroxyl group, shifting λmax to 280 nm. The lone pairs on nitrogen in the -NH₂ group have a strong electron-donating effect, further stabilizing the excited state and reducing the energy required for the transition.
Effect of Conjugation and Solvents on Transitions of Organic Molecules
Effect of Conjugation
Conjugation refers to the overlap of p-orbitals across adjacent double bonds, allowing for delocalization of π-electrons. This delocalization has significant effects on the absorption properties of molecules.
-
Shift in Wavelength:
- When double bonds (chromophores) in a molecule are conjugated, the absorption spectrum shifts toward longer wavelengths (a phenomenon known as a bathochromic shift).
- For example, in compound ‘A’, the conjugated double bonds result in a higher maximum wavelength (λmax) compared to compound ‘B’, which contains non-conjugated double bonds.
-
Energy Considerations:
-
The energy (E) of the transition between orbitals is given by the equation:
-
As conjugation increases, the energy (E) required for electronic transitions decreases. Consequently, the wavelength (λmax) at which absorption occurs increases.
-
Therefore, a greater extent of conjugation results in lower transition energies and higher λmax values.
-
Effect of Solvent
The solvent in which a molecule is dissolved can significantly influence its electronic transitions and absorption characteristics.
-
Polar Solvents and π → π* Transitions:
-
In polar solvents, π → π* transitions experience a red shift (shift to longer wavelengths). This occurs because the dipole interactions with polar solvent molecules stabilize the excited state (π*), lowering its energy compared to the ground state.
-
The stabilization effect can be attributed to hydrogen bonding or dipole-dipole interactions, which effectively lower the energy gap between the ground and excited states.
-
-
Polar Solvents and n → π* Transitions:
-
Conversely, n → π* transitions in polar solvents may exhibit a blue shift (shift to shorter wavelengths). This is due to the stabilization of the ground state (n) by dipole interactions, resulting in a decrease in its energy relative to the excited state.
-
In this case, the n electrons experience greater stabilization due to solvation effects, which increases the energy difference between the ground and excited states.
-
Applications of UV Spectroscopy
-
Extent of Conjugation:
- As the number of double bonds in a compound increases, the absorption shifts toward longer wavelengths. This helps in determining the level of conjugation in a molecule.
-
Distinction Between Conjugated and Non-conjugated Compounds:
-
UV spectroscopy can differentiate between conjugated and non-conjugated compounds. In the below image, isomers A and B are shown, where A is conjugated and absorbs at a longer wavelength due to the transition compared to non-conjugated isomer B.
-
-
Study of Geometric Isomerism:
-
The image (below) shows how UV spectroscopy is used to study geometric isomers (cis and trans forms). Due to steric hindrance, the cis-isomer (A) absorbs at a lower wavelength (283 nm) compared to the trans-isomer (B), which absorbs at 296 nm. This is because steric hindrance destabilizes the molecule, increasing its energy and decreasing the wavelength of absorption.
-
-
Detection of Impurities:
- Impurities in a compound can be detected through UV spectroscopy as they often give additional peaks in the UV spectrum.
-
Concentration Measurement: UV spectroscopy is frequently used in quantitative analysis, following Beer-Lambert's law, which relates absorbance to concentration. This principle allows for the determination of the concentration of analytes in solution, making it a fundamental technique in analytical chemistry.